Embark on a captivating journey through the quantum world of Quantum Electrodynamics (QED) and quantum computation, unraveling the foundational principles, historical milestones, and practical applications that shape the future of technology. Join us in exploring the intricate dance between light and matter, as we delve into the cutting-edge research that is revolutionizing the realm of computing.
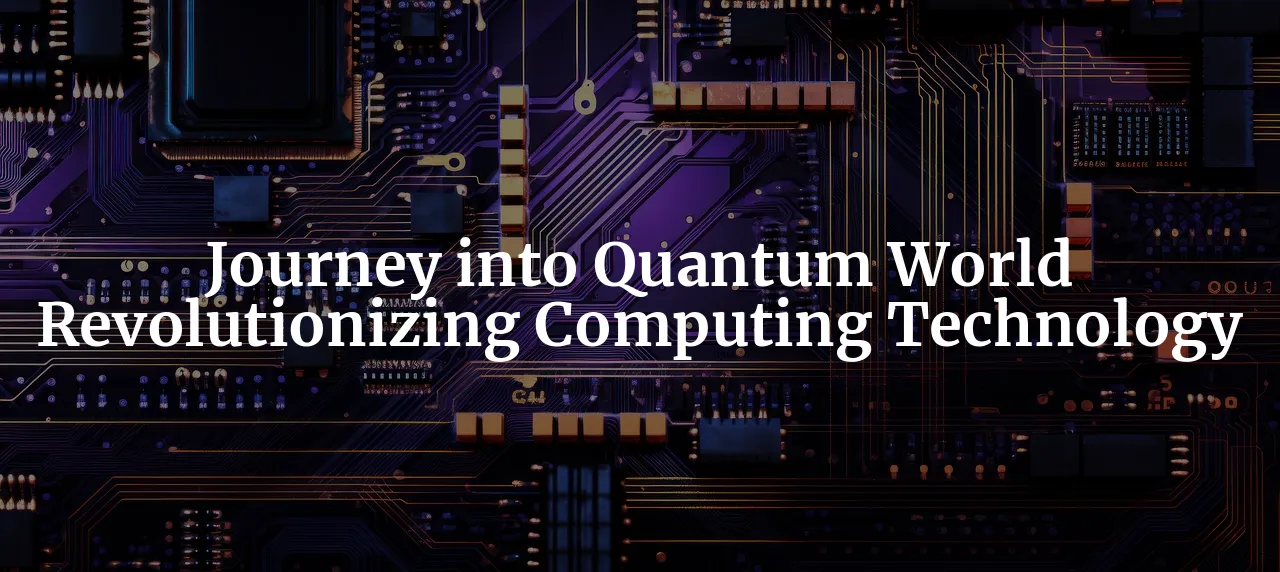
Introduction
In the realm of modern physics, quantum computation emerges as a revolutionary technology with the potential to solve complex problems far beyond the capabilities of classical computers. At the heart of this technological evolution lies Quantum Electrodynamics (QED), a fundamental theory that meticulously describes the interaction between light and matter at the quantum level 3. Understanding QED is not merely academic; it is a prerequisite for advancing quantum computing technologies, enabling scientists and engineers to manipulate qubits—the basic units of quantum information—with unprecedented precision 2.
The journey of QED from its classical roots to its current status as a quantum field theory is both fascinating and instructive. It illustrates the transition from a macroscopic understanding of electromagnetic phenomena to a quantum framework that accounts for the behavior of electrons and photons at the smallest scales 3. This evolution underscores the importance of QED in facilitating precise control over quantum states, thereby laying the foundation for quantum information processing and computation 1.
This article aims to explore why QED is indispensable for quantum computation, offering insights from historical, technical, and practical perspectives. By delving into the foundations of Quantum Electrodynamics, we will uncover the principles that enable the manipulation of qubits and highlight the role of QED in the burgeoning field of quantum computing. Join us as we unveil the quantum world, where the interplay of light and matter opens up new horizons for technology and science.
The Foundations of Quantum Electrodynamics
At its core, Quantum Electrodynamics (QED) is the relativistic quantum field theory of electrodynamics, providing a comprehensive mathematical framework for understanding the interactions between electrically charged particles and the electromagnetic field 3 ↗ ↗. The significance of the Dirac equation and the Klein-Gordon equation in QED cannot be overstated, as these equations describe the behavior of electrons and photons, respectively, with remarkable precision 3.
The Dirac equation, a cornerstone of QED, accounts for the relativistic effects of spin-1/2 particles, such as electrons and positrons, offering insights into their quantum behavior:
\[ i\hbar \frac{\partial \Psi}{\partial t} = c \boldsymbol{\alpha} \cdot \mathbf{p}c \beta \Psi + mc^2 \beta \Psi \mathrm{\ Dirac\ Equation} \]
Similarly, the Klein-Gordon equation extends the Schrödinger equation to relativistic speeds, applicable to bosons like the photon:
\[ \left(\frac{1}{c^2} \frac{\partial^2}{\partial t^2} - \nabla^2 + \frac{m^2c^2}{\hbar^2}\right)\psi = 0 \mathrm{\ Klein-Gordon\ Equation}\]
These equations are instrumental in predicting the outcomes of quantum interactions with high accuracy.
One of the most intriguing aspects of QED is the concept of virtual photons, which mediate forces between charged particles. Unlike real photons, virtual photons are not directly observable but play a crucial role in the exchange of forces at the quantum level. This exchange is visualized as happening through the emission and absorption of virtual photons, providing a quantum mechanical explanation for electromagnetic interactions that classical theories could not fully account for 3.
The precision and predictive power of QED have made it one of the most stringently tested theories in physics. Through experiments and measurements, such as the anomalous magnetic moment of the electron, QED has demonstrated an extraordinary level of accuracy. The most precise value of the electromagnetic fine-structure constant, (\alpha), confirms QED's predictions to within ten parts in a billion 5. This level of precision not only validates the theory but also highlights its importance in understanding the quantum world.
Historically, the development of QED is marked by the contributions of several key figures, including Feynman, Schwinger, and Tomonaga, whose work laid the groundwork for the theory as we know it today. Their collective efforts in formulating the interactions of light and matter within a quantum framework have paved the way for advancements in quantum computing and beyond.
The foundations of Quantum Electrodynamics provide the essential theoretical underpinnings for understanding and manipulating the quantum world. As we venture further into the realm of quantum computation, the principles of QED will continue to guide us, enabling the development of technologies that were once thought to be the realm of science fiction.
Quantum Computation and Qubits
Quantum computation represents a groundbreaking shift from classical computing, leveraging the principles of quantum mechanics to process information in ways previously thought impossible. At the heart of this revolutionary approach are qubits, or quantum bits, which differ fundamentally from their classical counterparts ↗. Unlike classical bits that exist in a state of either 0 or 1, qubits can exist in superpositions of states, embodying the quantum principle that particles can be in multiple states at once 2.
Qubits are manipulated using quantum logic gates, which, akin to classical logic gates, perform operations on qubits to execute computations. However, quantum gates exploit quantum phenomena such as superposition and entanglement to perform complex calculations more efficiently than classical gates 6. Superconducting circuits based on Josephson junctions have emerged as practical realizations of qubits, showcasing the ability to control qubit interactions at the quantum level with high precision 2. This control is crucial for quantum computation, as it enables the precise manipulation of qubits necessary for algorithm execution.
The landscape of superconducting qubits is diverse, with several types including charge qubits, flux qubits, and transmon qubits. Each type has its unique properties and advantages, but they all share the common feature of large dipole moments, making them suitable for coupling with the light field in circuit Quantum Electrodynamics (QED) settings 1. This interaction between qubits and photons is essential for quantum information processing, as it allows for the encoding, manipulation, and reading of quantum information.
Recent experimental advancements have significantly bolstered qubit technology. Notably, the demonstration of entanglement between qubits and the successful execution of gate operations have marked critical milestones. These achievements underscore the potential of superconducting circuits for quantum computation, paving the way for the development of more complex quantum systems capable of tackling problems beyond the reach of classical computers 6.
Circuit Quantum Electrodynamics (cQED)
Circuit Quantum Electrodynamics (cQED) delves into the intricate interaction between light (photons) and matter (qubits) within on-chip resonators. This field of study is pivotal for quantum computation as it provides a framework for the coherent coupling of single photons with artificial atoms, thereby enabling the manipulation of quantum information 1.
In cQED, superconducting coplanar waveguide microwave resonators are employed, which are typically made from materials such as aluminium or niobium and dielectrics like silicon or sapphire. These resonators are designed to support the coherent interaction between microwave photons and superconducting qubits, facilitating the exchange of quantum information 1.
The theoretical backbone of cQED is the Jaynes-Cummings model, which offers a full quantum mechanical description of the matter-light interaction. The Jaynes-Cummings model describes the interaction between a two-level quantum system (e.g., an atom with two energy levels) and a quantized electromagnetic field (a single mode of a cavity). The Hamiltonian for the Jaynes-Cummings model is given by:
\[ H = \hbar \omega_0 a^\dagger a + \frac{\hbar \omega_1}{2} \sigma_z + \hbar g (a \sigma_+ + a^\dagger \sigma_-) \mathrm{\ Jaynes-Cummings\ Model} \]
Here, \(a^\dagger\) and \(a\) are the creation and annihilation operators for the cavity mode, \(\sigma_z\) is the Pauli z-matrix for the two-level system, and \(\sigma_{+/-}\) are the raising and lowering operators for the two-level system, respectively. The \(\omega\) parameters, and g are the frequencies of the cavity mode and the two-level system, and the coupling strength, respectively.
This model is particularly significant as it includes cavity, atomic, and interaction terms, providing insights into how photons and qubits interact at the quantum level ↗. One of the model's key predictions is the lifting of the degeneracy of photon number states and atomic states, leading to the formation of dressed states. These states are energetically split by a detuning factor, a phenomenon that has been experimentally observed and is crucial for quantum computation 1.
Circuit QED has achieved remarkable milestones, including deterministic gate teleportation and operations on multiple qubits. These achievements are indicative of the field's potential to realize practical quantum computing systems. A critical factor in these successes is the ability to reach the strong coupling regime in cQED, where the coupling strength between qubits and resonators significantly exceeds the cavity loss rate and decoherence rate. This regime is essential for effective quantum computation, as it ensures that quantum information can be processed and stored with high fidelity 1.
Furthermore, the complementary relationship between circuit QED and cavity QED enriches our understanding of quantum physics. Cavity quantum electrodynamics describes strong coupling between cavity photons and single optical emitters, therefore cavity QED leads to correlation effects between emitted photons, resulting in non-classical light 4 ↗. Both fields, while focusing on different systems, provide valuable insights into the same underlying quantum phenomena, showcasing the versatility and power of quantum electrodynamics in advancing quantum computation 2.
The interplay of qubits and photons within the framework of circuit QED is forging new paths in quantum computation. By harnessing the principles of quantum mechanics, researchers are inching closer to realizing quantum computers capable of solving complex problems that are currently intractable for classical computers, marking a new era in computational science.
Historical Milestones and Key Figures
The journey of Quantum Electrodynamics (QED) from its conceptual beginnings to its pivotal role in quantum computing is a fascinating saga of intellectual triumph. The roots of QED can be traced back to the early 20th century, when the foundations of quantum mechanics were being laid. The development of QED, as we understand it today, was a monumental leap in our understanding of the quantum world, providing a mathematical framework that describes how light and matter interact. This framework has been crucial in the evolution of quantum computing.
A significant milestone in the history of QED was the pioneering work of John M. Martinis, Michel Devoret, and John Clarke in 1985, who demonstrated the quantum behavior of Josephson junction circuits. This breakthrough laid the groundwork for the development of superconducting qubits, which are at the heart of many quantum computing architectures today 2. Their work showed that it was possible to control and manipulate quantum states in solid-state devices, opening up new avenues for quantum computation.
Further advancements in the field were made by Blais et al., who developed cavity QED for superconducting electrical circuits. This development provided a robust architecture for quantum computing, enabling the coherent coupling of qubits with microwave photons, a critical capability for quantum information processing 1. The work of Blais and his colleagues has been instrumental in moving quantum computing from theoretical models to practical, scalable architectures.
Another landmark achievement was the demonstration of strong coupling in circuit QED by Wallraff et al. This experiment showed that a single photon could be strongly coupled to a superconducting qubit, a key requirement for quantum computation, as it allows for the coherent transfer of quantum information between qubits and photons 1. This achievement was a significant step forward in the quest to build a quantum computer.
Koch et al.'s design of charge-insensitive qubits was another critical development in the field. By making qubits less sensitive to charge noise, they improved the stability and coherence times of qubits, addressing one of the major challenges in quantum computing 1. This innovation has had a lasting impact on the design of superconducting qubits, making them more practical for quantum computation.
The combined efforts of theorists and experimentalists have propelled the field of quantum computing forward, leveraging the principles of QED to develop new technologies and architectures. The historical milestones and key figures in the development of QED have laid the foundation for the quantum computers of the future, promising to revolutionize computing by solving problems that are intractable for classical computers.
Practical Applications and Future Prospects
The practical applications of quantum computing are vast and varied, ranging from cryptography and drug discovery to solving complex optimization problems. The unique capabilities of quantum computers, such as parallel processing and the ability to simulate quantum systems, make them ideally suited for tasks that are beyond the reach of classical computers.
In the field of cryptography, quantum computing poses both a challenge and an opportunity. Quantum algorithms, such as Shor's algorithm, can break many of the cryptographic schemes currently in use, posing a threat to digital security. However, quantum computing also enables the development of quantum cryptography, which promises to be secure against attacks by quantum computers.
Drug discovery is another area where quantum computing has the potential to make a significant impact. Simulating the quantum behavior of molecules is a challenging task for classical computers but is naturally suited to quantum computers. This capability could revolutionize the pharmaceutical industry by making the drug development process faster and more cost-effective.
Quantum computing also holds promise for solving complex optimization problems, which have applications in logistics, finance, and artificial intelligence. Quantum algorithms have the potential to find solutions to these problems more efficiently than their classical counterparts, leading to significant improvements in these fields.
The future prospects of quantum computing are closely tied to advancements in QED and the development of quantum technologies. Ongoing research is focused on improving qubit coherence times, increasing the scalability of quantum computing architectures, and developing hybrid systems that integrate circuit QED with other quantum systems 6. These efforts aim to overcome the current challenges facing quantum computing, such as decoherence and the need for low-temperature environments.
The economic and political implications of quantum computing are profound, with national security concerns and the race for technological supremacy driving investment in quantum research. The interdisciplinary nature of quantum computing research, involving physics, computer science, and engineering, underscores the collaborative effort required to realize the full potential of this technology.
The practical applications and future prospects of quantum computing are vast, with the potential to revolutionize various fields. The advancements in QED and quantum technologies are paving the way for the development of practical quantum computers, promising to unlock new capabilities and solve problems that are currently intractable. As research in this field continues to advance, the future of quantum computing looks bright, with endless possibilities on the horizon.
Conclusion
The journey through the quantum world of Quantum Electrodynamics (QED) has illuminated the path towards the realization of quantum computation. The foundational principles of QED, rooted in the intricate dance between light and matter at the quantum level, have paved the way for the development of revolutionary technologies that transcend the limitations of classical computing. From the theoretical underpinnings of the Dirac and Klein-Gordon equations to the practical applications of qubits and quantum logic gates, QED has been instrumental in shaping the landscape of quantum computing.
The historical milestones and key figures in the field of QED have left an indelible mark on the evolution of quantum computing. Visionaries like Feynman, Schwinger, and Tomonaga laid the groundwork for our current understanding of QED, while modern pioneers such as Martinis, Devoret, and Clarke have pushed the boundaries of quantum technologies through experimental breakthroughs. Their collective efforts have propelled quantum computing from theoretical concepts to practical applications, setting the stage for a new era of computational science.
Looking ahead, the practical applications and future prospects of quantum computing are boundless. From revolutionizing cryptography and drug discovery to solving complex optimization problems, quantum computers hold the promise of transforming industries and society at large. As research continues to advance in QED and quantum technologies, the prospects for practical quantum computers grow brighter, offering endless possibilities for innovation and discovery.
As undergraduate and graduate students of physics, the realm of quantum electrodynamics and quantum computation beckons with exciting challenges and opportunities. By delving into the intricacies of QED and its applications in quantum computing, you can contribute to the cutting-edge research that is shaping the future of technology. Embrace the interdisciplinary nature of quantum computing, collaborate across disciplines, and push the boundaries of knowledge to unlock the full potential of this transformative field.
In closing, the fusion of Quantum Electrodynamics and quantum computation represents a paradigm shift in the realm of computing. By harnessing the power of QED, we stand on the cusp of a new era where quantum computers will revolutionize the way we solve problems, process information, and understand the universe. Let us venture forth with curiosity, determination, and a shared vision for a future where the quantum world unfolds its mysteries and possibilities before us.
References
-
Circuit quantum electrodynamics - Wikipedia - en.wikipedia.org ↩↩↩↩↩↩↩↩↩
-
Coupled high-Q micro-cavities - www.ksop.kit.edu ↩
-
Precision tests of QED - Wikipedia - en.wikipedia.org ↩
-
Quantum information processing and quantum optics with circuit quantum electrodynamics - www.nature.com ↩↩↩